Published on 2024/11/20 Research powered by Mightex’s Polygon1000
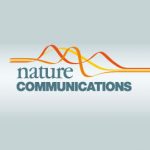
Despite the immaturity of the animal cortex at birth, one property of interest is present: the columnar, modular organization of activity in the cortex. This means the cortex naturally organizes its neural activity into alternating bands of active and inactive regions, creating a predictable spatial pattern.
While this phenomenon has been investigated in prior research, it has yet to be determined what the underlying structural and connective properties are that support this property. A primary theory states that this property results from a combination of lateral inhibition and local excitation. Under this paradigm, neurons, when activated, send excitatory signals to nearby neurons within their given column but send inhibitory signals to those nearby neurons outside their given column. In this way, by combining columnar signal propagation with lateral inhibition, a spatial periodic activity pattern would be reinforced in the cortex.
In their Nature Communications publication titled “Self-organization of modular activity in immature cortical networks”, Mulholland, Kaschube, and Smith (2024) investigate the mechanism behind this cortical property. To determine if indeed lateral inhibition and local excitation are the mechanisms underlying the spatially periodic quality of activity present in the cortex, the researchers first referenced mathematical models of lateral inhibition and local excitation and determined that any neural system with both lateral inhibition and local excitation would have the following four properties:
- Even with a random stimulus, the neural tissue will spontaneously organize its activity into a regular, predictable spatial pattern. (Figure 1a).
- The tissue will consistently produce activity patterns at a specific characteristic frequency, which remains stable across different trials and animals.
- If the tissue is stimulated at the characteristic frequency, then the phase of the activity that results from the stimulus should align with the phase of the stimulation (Figure 1b).
- If the tissue is stimulated at a non-characteristic frequency, then the resultant activity will be some intermediate between the characteristic frequency of the structure and the stimulation frequency (Figure 1c).
Figure 1. Properties of a system driven by local excitation and lateral inhibition. The die represents random variation that is present in every neural process. (a) The researchers theorize that with a random or generic stimulus, the resultant activity should take on a spatially periodic form. ⋀ represents the characteristic frequency of the tissue.(b) The researchers theorize that, if a stimulus is presented to the tissue that is the same frequency as the characteristic frequency of the tissue then the phase of the presented stimulus and the resultant activity will be the same. λI and λO represent the frequency of the stimulus and the resultant activity respectively. (c) The researchers theorize that if a stimulus is presented to the tissue that is not the characteristic frequency then the resultant activity will have some intermediate frequency in between the characteristic frequency of the tissue and the frequency of the stimulus.
To test the theory that lateral inhibition and local excitation are the mechanisms supporting the modular, columnar activity present in cortical tissue, Mulholland, Kaschube, and Smith tested each of the 4 properties listed above. The researchers used simultaneous calcium imaging (GCaMP6s) and optogenetics (ChrimsonR) for full optical control and imaging of cortical tissue in immature ferrets (Figure 2).
Figure 2. Surgical and experimental procedure. Immature ferrets were, on post-natal days 10-15, injected with GCaMP6s and ChrimsonR. Then, on post-natal days 23-29, simultaneous optogenetic and imaging experiments were run.
After infecting tissue in the 2nd and 3rd layers of the visual cortex, the researchers headfixed the animals and used high power LED light sources to image and a 590nm laser to stimulate the tissue. In order to pattern the laser illumination onto the tissue for stimulation, a Mightex Polygon1000 Pattern Illuminator was used.
In a first experiment designed to investigate property 1 and 2, the researchers stimulated the visual cortex in an unorganized manner. In response, the tissue activated in a periodic pattern. Indeed, they further observed that, upon repeated activation of a given animal’s visual cortex, the frequency of the pattern varied little both within and between animals. These findings are consistent with properties 1 and 2 (Figure 3).
Figure 3. A measurement of modularity, or columnar and organized the activity within the tissue. During broad optogenetic stimulation, the modularity of the activity increased significantly for all animals compared to visual cortex activity prior to stimulation.
In a second experiment designed to investigate property 3, the researchers used the Polygon1000 Pattern Illuminator to stimulate the tissue optogenetically. The researchers used data from the previous experiment to find the characteristic spatial frequency of each animal’s visual cortex and stimulated their cortex at that same frequency. The researchers found that the phase of the stimulation and the phase of the activity pattern lined up (Figure 4). This finding is consistent with property 3.
Figure 4. Similarity of the stimulus to the response in the visual cortex. In this experiment, researchers stimulated the visual cortex with a pattern that had the approximate endogenous frequency of the tissue. They then compared, in the stim condition, the stimulation pattern the resultant activity. In the shuffle condition, they compared the stimulation pattern to a random activity pattern recorded during a different session. The researchers found that the specific pattern that is stimulated is highly similar to the resultant activity. The filled in black triangle is the average value of the stim data while the thick X is the average value of the shuffle data.
In a third experiment designed to investigate property 4, the researchers patterned light at a non-characteristic frequency onto the cortex. They found that the activity pattern that resulted from stimulation was an intermediate waveform; its frequency was between the characteristic frequency of the tissue and the frequency of the stimulation (Figure 5). This finding is consistent with property 4.
Figure 5. Effect of varying input wavelength on output wavelength. As the input wavelength varies from the characteristic wavelength of the tissue (blue line), the output wavelength of the system drifts from the characteristic waveform.
Altogether the researchers, using an all optical approach for both imaging and stimulation, were able to test the visual cortex of immature ferrets to see if their activity patterns were consistent with those that should appear in systems that use both lateral inhibition and local excitation. They found that the visual cortex performed consistently with those properties. Mulholland, Kaschube, and Smith argue that these findings further evidence the theory that lateral inhibition and local excitation properties are genetically encoded in the cortex.
Joe Banning, Applications Specialist at Mightex
To read the full publication, please click here.