Published on 2025/04/14 Research powered by Mightex’s Polygon1000
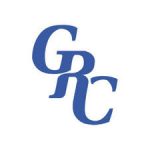
This research was recently presented by Jihwan Lee at the ASCB (2024) in San Diego,CA,USA and was supported by a Mightex Travel Award to promote scientific communication and sharing of research using the Mightex Polygon. Congratulations to Jihwan Lee! We can’t wait to see more from this exciting project!
Introduction
Metagenomic screening and protein engineering have produced a broad color palette of fluorescent proteins (FPs) to visualize biological events and structures. However, many FPs rapidly photobleach under repeated or prolonged illumination, limiting the duration and temporal resolution in live cell microscopy. Rapid photobleaching is especially pronounced in high-power
and long-exposure techniques such as super-resolution1, single-molecule2,3, and voltage imaging 4. StayGold, a highly photostable green FP reported recently, facilitates prolonged imaging experiments 5–8. However, the photolability of FPs in other spectral ranges persists, limiting extended multi-spectral imaging. Notably, yellow FPs (YFPs) typically photobleach faster than counterparts from other spectral classes. Given the widespread use of YFPs in fluorescent biosensors 9–11 and multi-spectral imaging 12–14, enhancing their photostability is imperative.
Results
Single-cell screening platform identifies two bright YFPs with improved photostability
We sought to address the relative photolability of current YFPs via high-throughput protein engineering. Screening was performed using SPOTlight, our pooled single-cell screening platform 15 (Fig. 1). We randomly mutagenized mGold 15, the most photostable monomeric YFP we previously derived from mVenus 15 (Fig. 1a, step 1). We screened the resulting libraries in yeast cells, evaluating the brightness and photostability of 1,125,438 cells representing 204,987 variants across 7 rounds of screening (Fig. 1a, step 2). In each screening round, we optically labeled 200-
400 single variants (Fig. 1a, step 3→4) for retrieval via Fluorescence Activated Cell Sorting (FACS). Using the Mightex Polygon 1000 digital micromirror device was critical because it enabled precise single-cell optical labeling. Compared to DMD from another company, Mightex’s DMD provided greater illumination strength on the sample plane, enabling faster optical tagging. Optically labeled cells were then grown into colonies on agar plates, picked, expanded, and plated onto multi-well plates (Fig. 1a, step 4). We performed population-level validation by quantifying the mean brightness and photostability of thousands of cells per variant, and the top 5-10 variants were sequenced.
Characterization and application of mGold2s and mGold2t
The two best variants based on brightness and photostability were named mGold2s and mGold2t. When we imaged live mammalian cells expressing mGold2s and mGold2t using continuous widefield illumination of 520/5 nm (central wavelength/spectral width) light at 2.4 mW/mm2 for 1 hour, mGold2s and mGold2t retained 50% and 43% of their original fluorescence, corresponding to photobleaching half-lives of 60 and 42 minutes, respectively (Fig. 1b). In contrast, mVenus and mGold rapidly photobleached with half-lives of 3 and 12 minutes, respectively. mGold2s and mGold2t showed different photobleaching patterns: mGold2t initially photobleached more rapidly than mGold2s, but this trend reversed at later time points (Fig. 1c).
To demonstrate the utility of the photostable mGold2 variants, we assessed the performance of mGold2s and mGold2t in super-resolution imaging using structure-illuminated microscopy (SIM). We imaged actin filaments in COS-7 cells expressing YFP-LifeAct fusions at a rate of 9 Hz for ~1.5 minutes, using a 488-nm laser at 95 mW/mm2. Each time point comprised nine raw frames
reconstructed into a single SIM image. The resolution of SIM images was further enhanced through sparse deconvolution 16 (Fig. 1c–d). mGold2s and mGold2t maintained over 80% of their initial fluorescence, significantly outperforming mVenus, which retained only about 25% (Fig. 2e). mGold2s enabled visualization of actin dynamics similar to the “actin blip” dynamics described previously 17 (Fig. 2f). This dynamic event involves actin punctum formation at the network node followed by disassembly of the punctum and can detected as a spike in fluorescence intensity. These dynamics could not be observed using photolabile mVenus because of rapid photobleaching.
Conclusion
We anticipate that these new variants will set a new gold standard in the realm of monomeric YFPs, offering extended imaging durations and improved temporal resolution for biological research and biotechnological applications.
Figure 1. Development, characterization, and application of mGold2 YFPs. (a) SPOTlight screening workflow used to develop bright and photostable YFP variants. (b) mGold2s and mGold2t expressed in HEK293A cells retained around half of their fluorescence after continuous illumination of 520/5-n light at 2.4 mW/mm2 for 1 hr. (c) YFP-LifeAct fusions were expressed in COS-7 cells to visualize actin filaments. (d) Imaging workflow. (e) mGold2s and mGold2t shoed greater photostability than mVenus under TIRF-SIM with 488 nm light at 95 mW/mm2. Bottom graph: Traces denoted the mean, and the thin shaded area are the 95% CI. n = 40-50 regions of interest across 10 cells. (f) mGold2s enabled extended visualization of actin dynamics. The arrow denotes an actin blip.
References
1. Lambert, T. J. & Waters, J. C. Navigating challenges in the application of superresolution microscopy. J. Cell Biol. 216, 53–63 (2016).
2. Schirripa Spagnolo, C. & Luin, S. Choosing the Probe for Single-Molecule Fluorescence Microscopy. Int. J. Mol. Sci. 23, 14949 (2022).
3. Jain, A. et al. Probing cellular protein complexes using single-molecule pull-down. Nature 473, 484–488 (2011).
4. Yang, H. H. & St-Pierre, F. Genetically Encoded Voltage Indicators: Opportunities and Challenges. J. Neurosci. 36, 9977–9989 (2016).
5. Hirano, M. et al. A highly photostable and bright green fluorescent protein. Nat. Biotechnol. 40, 1132–1142 (2022).
6. Ando, R. et al. StayGold variants for molecular fusion and membrane-targeting applications. Nat. Methods (2023) doi:10.1038/s41592-023-02085-6.
7. Ivorra-Molla, E. et al. A monomeric StayGold fluorescent protein. Nat. Biotechnol. (2023) doi:10.1038/s41587-023-02018-w.
8. Zhang, H. et al. Bright and stable monomeric green fluorescent protein derived from StayGold. Nat. Methods (2024) doi:10.1038/s41592-024-02203-y.
9. Tutol, J. N. et al. Engineering the ChlorON Series: Turn-On Fluorescent Protein Sensors for Imaging Labile Chloride in Living Cells. ACS Cent. Sci. 10, 77–86 (2024). 10. Ni, Q. et al. Signaling diversity of PKA achieved via a Ca2+-cAMP-PKA oscillatory circuit. Nat. Chem. Biol. 7, 34–40 (2011).
10. Ponsioen, B. et al. Detecting cAMP-induced Epac activation by fluorescence resonance energy transfer: Epac as a novel cAMP indicator. EMBO Rep. 5, 1176–1180 (2004). 12. Cohen, S., Valm, A. M. & Lippincott-Schwartz, J. Multispectral live-cell imaging. Curr. Protoc. Cell Biol. 79, e46 (2018).
11. Livet, J. et al. Transgenic strategies for combinatorial expression of fluorescent proteins in the nervous system. Nature 450, 56–62 (2007).
12. Cai, D., Cohen, K. B., Luo, T., Lichtman, J. W. & Sanes, J. R. Improved tools for the Brainbow toolbox. Nat. Methods 10, 540–547 (2013).
13. Lee, J. et al. Versatile phenotype-activated cell sorting. Sci. Adv. 6, eabb7438 (2020). 16. Zhao, W. et al. Sparse deconvolution improves the resolution of live-cell super-resolution fluorescence microscopy. Nat. Biotechnol. 40, 606–617 (2022).
14. Mo, Y. et al. Quantitative structured illumination microscopy via a physical model-based background filtering algorithm reveals actin dynamics. Nat. Commun. 14, 3089 (2023).
Author: Jihwan Lee
Bio: Temporary post-doctoral associate at St-Pierre Lab, Department of Neuroscience, Baylor College of Medicine, Rice University. Advisor: Dr. François St-Pierre.